What the Tiny Cluster of Brain Cells in My Lab Are Telling Me
NRI Co-Director, Dr. Kenneth Kosik has created organoids that, surprisingly, have a lot to say about how the brain works.
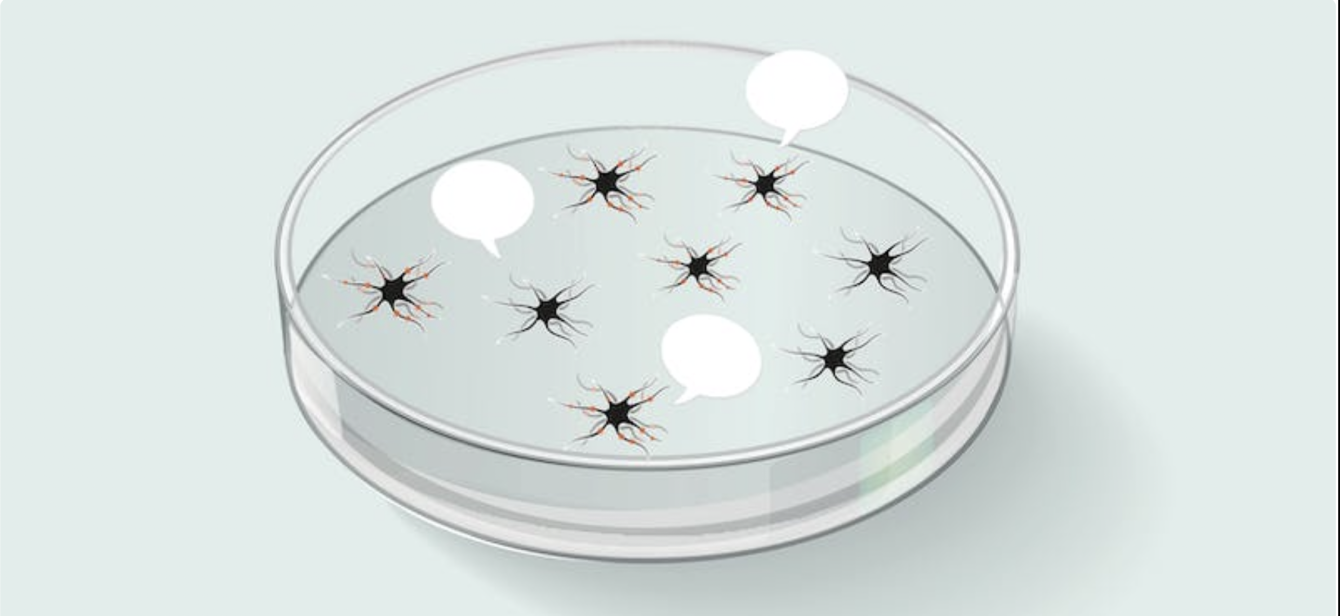
My feeling was nothing less than elation. Tal Sharf, one of my post-doctoral fellows, called me from my neurobiology lab at the University of California, Santa Barbara, with the news that a tiny blob of tissue resembling a brain was talking in the only way it could. It was producing indecipherable electrical squiggles on a computer screen.
I was in a planning meeting for a class designed to link neuroscience and the humanities. My colleagues, professors in the art and music departments, were puzzled and curious about the enthusiasm written on my face. Wanting to keep the discussion grounded in science, not science fiction, I began to explain.
Was there a fragment of consciousness in the tissue speck, a whisper from the genes of the donor?
The tissue blob was called a brain organoid. It began as a biopsy from the skin of a donor and was planted on a culture dish. Four critical genes encased in a dagger-like microscopic vehicle were driven into the skin cells to erase their memories of having been skin, and then reprogrammed as stem cells. Stem cells, the source of the diversity among cell types in the body, from liver cells to hair follicles, from lung cells to lacrimal cells, have the potential to become any cell type in the human body.
My postdocs and I gently prodded the cells to become brain cells by providing the necessary chemical growth conditions in their fluid bath that guided them toward a neuronal fate. We injected a drop of these cells inside a gel-like matrix so they could grow into a three- dimensional shape rather than grow conventionally on a flat surface like characters in Flatland. Once in this new dimension, the diverse cell types shape themselves into the layered organization that loosely resembles, in miniature, a developing human brain, filled with tentacular axons coursing among the branches and twigs of tree-like dendrites dotted with synapses.
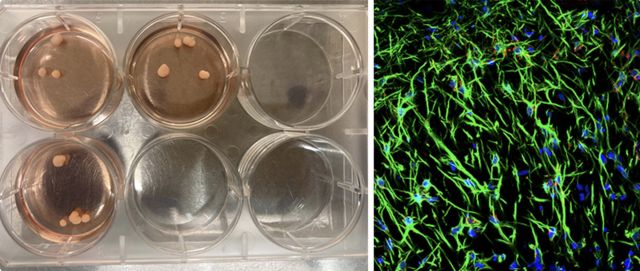
My humanities colleagues were fascinated. Was there a fragment of consciousness locked inside this tissue speck, a whisper from the genes of the donor? I momentarily ignored such fantastical misconceptions. My project was motivated by the brain as unknown territory, and the unknown has an allure that lies deep in human nature. In particular, we had undertaken a search for a core network, an intrinsic coding system in the organoid that could be applicable to the human brain. Less lofty, more practical motives also drove my interest. Organoids provide a platform for drug discovery and disease modeling. So when the organoids started talking in the language of electrical signals, we were eager to learn what they were saying.
In my lab, the brain organoids are specks of tissue floating in a Petri dish. Once sliced open and stained, a panoply of neurons, astrocytes, and oligodendrocytes appear through the ocular of a microscope. Over the next few months, the organoid growth paralleled the development of a human cerebral cortex fallen into an Alice in Wonderland rabbit hole in which everything was very small. And, without a blood supply, it can never grow much larger.
To listen in on the electrical chatter, we carefully lifted this quinoa-grain-sized bit of tissue from the fluid and seated it on a surface densely packed with electrodes, more than 26,000 of them, on about two square millimeters of real estate covered in a fluid of nutrients that approximates cerebral spinal fluid. When a neuron fires, it sends a tiny voltage pulse into the fluid that is detected by nearby electrodes and visualized as a blip on a computer screen. These blips are called spikes. Of nearly a million neurons in the organoid (compared to 86 billion in the human brain), the electrodes capture a few hundred of them.
In the actual brain, when thousands of spikes synchronize their rhythms, they can generate brain states such as attention, mind-wandering, or sleeping. Pioneering neuroscientist Györgi Buzáki compared this to the sounds from a football stadium in which individual voices cannot be discerned, but the collective roar of the crowd signals important events such as a goal. However, the rhythms of the brain are more complex. Intrinsic rhythms at multiple frequencies oscillate through the brain, collide and disperse, like the wake from a motorboat angling through ocean waves.
The organoid growth paralleled the development of a brain fallen into Alice in Wonderland.
In his 1949 book, Organization of Behavior, Donald Hebb, a founder of modern neuropsychology, hypothesized that strongly interconnected neurons can represent distinct complex cognitive processes such as memory, abstraction, and decision-making. Later work identified these assemblies as large groups of collectively firing neurons that generate the rhythms. A mathematical method called Fourier Transform converts this chatter into a wave spectrum of individual frequencies, some fast, some slow.
Our thrill of discovery grew when we captured sets of spikes aligned with certain wave frequencies. In the brain, these alignments between spikes and waves, called phase-locking, wire together a set of spikes into a connected network and assign a meaning to the network as a representation of an experience. But there was no experience to spur the phase-locking! There was just an intrinsically generated signal coming from a Petri dish inside a dark incubator signifying nothing more than a wiring diagram, a spontaneously emergent framework potentially ready to encode experience.
Unlike an organoid, humans and all animals develop their brains concurrently with their bodies. From the moment the developing nervous system senses the rest of its body, it begins to register incoming signals such as a heartbeat and gut motility for computing predictions and implementing actions such as kicking and squirming. Spontaneous activity in organoids, in the absence of any stimulus from a body, and absent any feedback from a motor output to muscles, runs through its paces without even a scintilla of expectation that its signals might inform a body. It doesn’t simply lack a body in the way an amputated arm leaves its phantom trace in the brain. Rather, it spontaneously and inherently develops the appearance of a framework to communicate to a body that never arrives, that is not even conceived.
Our brain organoids seemed to reveal what the Nobel laureate John O’Keefe noted before organoids existed. O’Keefe wrote that brain systems “in which activity occurs in the absence of sensation serves as a physiologic scaffold capable of representing information from the external environment before it is experienced.” In a similar vein, Buzsáki had written the brain “starts out as a nonsensical dictionary. It comes with evolutionarily preserved, preconfigured internal syntactical rules that can generate a huge repertoire of neuronal patterns.” Those complex rhythms represent the innate machinery similar to the babble of infants, who toy with their vocal cords, tongue, and lips to make all possible sounds that increasingly narrow as the infant acquires the sounds of a specific language. Our brain organoids appeared to represent a piece of the operating system upon which experience could be superimposed. Were they in fact offering a peek, albeit imprecise, at the brain’s preconfigured internal syntactical rules?
I would like to say our experiments offered a glimpse of the brain’s internal syntax, the neural roots of thoughts and actions. But neural connectivity is a knotty problem. To visualize this organization, think of the dendrite as a tree with a complex branching pattern. Running through the branches are many axon-like electrical lines. Each line originates from a different neuron and a point where the line touches a tree branch is a synapse. Dendrites can have thousands of synapses that may or may not get activated by an incoming axon, but when enough of them are activated, the neuron spikes. The complexity lies in how the dendrite computes all of its inputs to make a single binary decision—to fire or not.
Organoids gave us insight into the operating system upon which experience is superimposed.
As a simplification, we computed the probability that if one neuron fires, say neuron A, a second neuron, say B, will fire within a very short time interval. In this way, we created a functional connectivity map. Over several minutes and for as long as a few hours, the connectivity map remains mostly stable in its structure. A strong connection occurs if the probability is high that B will fire after A fires. However, the famous principle that correlation is not causation leaves us uncertain as to whether A caused B to fire. A third neuron might induce the firing of both A and B. Causation also assumes that the cause precedes effect, but in the organoid, no time stamp indicating an event initializes the sequence, implying that over a longer interval B may cause A with a higher probability than the reverse.
By plotting the distribution of all the connection strengths, we see relatively few strong connections in a sea of weak connections. These connections become modified over time as the skeleton of more rigid information routes can draw upon a vast reservoir of weak connections to update the network. While the connectivity network is unattached to experience in the organoid, a similar network structure in the brain may encode recesses of memory fragments. As the connections that bind these memories spread through the network, those memories are bound by the stability of the network and will likely dissipate until forgotten in its outer reaches.
Our ongoing research attempts to induce a desired response in the organoid after it receives a specific electrical stimulus; for example, stimulating the same three electrodes multiple times. The electrical stimulus will activate other neurons in the organoid, and we expect will generate a reproducible signal that specifies the input signal. If these inputs are a proxy for authentic environmental inputs, a networked pattern of neuronal activity is detectable. That pattern, a complex representation of the input, potentially encodes the nuances and memories associated with an input and forms the physiological basis of a perception. For instance, a photon hitting the eye’s retina generates a neural firing pattern that arises from the input. To reckon with the complexity of the environment, the brain must generate multiple associations linked to memories for its inputs. The organoid, devoid of memories, creates empty network containers.
Insights from organoids in experiments like ours may one day help solve tricky clinical problems. (Information from organoids also promises to reduce the use of animal testing in drug design.) For example, the enormous amount of information stored in the brain shrinks in those with dementia, leaving affected individuals unable to fully comprehend their world. Sensory inputs to the brain—light, sound, touch, odors—convert features of the physical world to waveforms that ramify in the brain to generate memory, experience, and emotion. These inputs get incompletely or erroneously processed when synaptic connections are lost in conditions such as Alzheimer’s.
In the future, scientists could outfit an organoid with algorithms known as pattern completion, used in artificial intelligence. The brain-signal patterns associated with human memories, like a fleeting image of a night sky, or musical bits as if broadcast from spinning a radio dial, or partial identities of friends that wander in and out of dreams, can potentially be augmented in the organoid. The augmented organoid, transmitted back to the patient through a Metaverse-like headset, could potentially restore a lost or forgotten image and the memories and emotions associated with them. This would represent a synthetic consciousness with far greater versatility than a hard-wired computer chip.
So, back to my colleagues in the humanities. Does a brain organoid conceal some fragment of consciousness? Brain organoids themselves are not conscious for the simple reason that a core property of consciousness—abstraction—is not available to the organoid. Except for those marginal views of consciousness as a boundless, extracorporeal pan-psychic property, consciousness requires the generation of an abstraction derived from an interplay among our impressions of the sensory world and our motor responses.
Take a look at that red sphere-like object in a bowl on the dining room table. The light reflected from this object activates photoreceptors in the retina that send a signal to the brain carrying a wealth of information about its color, shape, size, and setting. After several years of experiencing the world, a firing pattern has emerged that corresponds to the word apple. The arbitrary sound of a word and its orthography correspond to a neural activity pattern. The firing pattern is also arbitrary because a different pattern in another brain could have the same association with the apple. The abstraction called apple comes from the link between a set of input spikes and the presence of the corresponding image in the world. The spikes are fundamentally what we see in the organoid; they are simply untethered to anything in the world.
Theories as to how abstraction pops out from circuitry abounds among consciousness researchers. None offer answers. The problem is so enormous that Roger Penrose, winner of the 2020 Nobel Prize in Physics, has said that whatever consciousness may be, it is not a computation, and suggested that a new mathematics might be required to solve it. The tractability of brain organoid circuitry provides one method for performing the detailed computations needed to chip away at what is happening inside the brain, and how that activity can be harnessed. No new math will come from my lab. So I may disappoint my humanities colleagues when I tell them that my brain organoids are silent on the subject of consciousness.
BY KENNETH S. KOSIK | Nautilus, November 15, 2022